How thermal storage can help with the climate crisis
Heating and cooling make up about 50% of our total energy consumption, but today’s global infrastructure is straining to keep up. Here’s how thermal storage may become a reliable and alternative energy storage method.
As the European energy crisis deepens, the upcoming 2022 winter is looking increasingly bleak. With the entire continent cut off from Russian oil and gas, people are literally struggling to keep their lights on and houses warm. Utilities already aren’t able to supply enough power, and when you factor in the impending heating needs over the coming months, things are looking increasingly difficult. European countries are considering capping energy bills, providing billions of dollars in aid, and even restarting coal plants.
And while the need for heat is most urgently felt in Europe right now, the entire world has huge heating and cooling needs that our current infrastructure is straining to keep up with. Heating and cooling make up about 50% of our total energy consumption. Electricity, in comparison, only makes up only about 20%. Some experts estimate that the heating and cooling market size is $1.4 trillion, and this figure is only getting larger. As the population grows, as the climate crisis warms the planet, and as developing nations electrify, we’re only going to need more heating and cooling capacity.
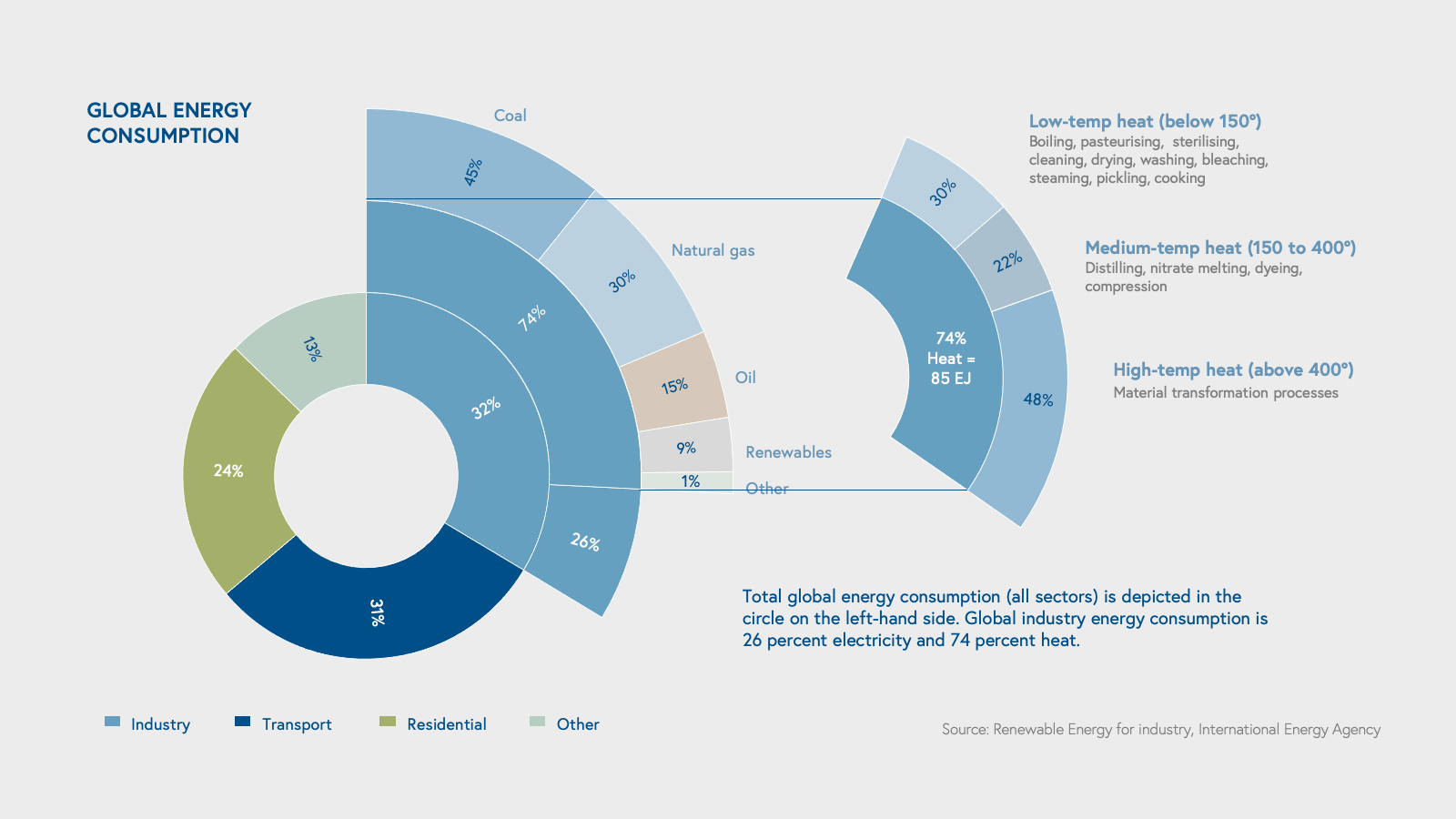
The problem is that heat today is predominantly generated by burning oil, coal, and natural gas. But we can’t afford to continue burning fossil fuels at this rate if we want to hit Net Zero by 2050. We need to decarbonize the heating and cooling industry.
Thankfully, we have a few options to meet this need. For instance, we’ve previously written about fourth-generation nuclear reactors, which generate heat at > 500° C. Other options include electrifying residential heat through heat pumps, drawing from and storing heat directly from the Earth’s core using geothermal energy, and developing cheap green hydrogen, which can supply heat at > 2000° C.
One solution we are excited about is thermal energy storage (TES). Thermal storage systems (aka “thermal batteries”) store excess heat and deploy it when needed. Most excitingly, TES has a high technological readiness level (TRL), and companies today are using thermal batteries to supply heat for a wide variety of applications. In this roadmap, we’ll explore the thermal storage market, its challenges, the different technologies being developed, and where this industry is heading.
The thermal storage market
Thermal storage systems can be used to tackle both the heat market and electricity market. Starting with heat, we noted the global heat market is large (on the order of trillions), but the market size is actually a bit more nuanced than that. The end application for the heat drives the required temperature, which in turn drives the usefulness of a given thermal storage system.
Consider, for example, heat used for industrial applications. Some industrial processes, such as cement making, require temperatures in excess of 1800° C. Others, like plastics processing, only require temperatures at around ~150° C. Residential heating and cooling requires temperatures less than 30° C. Unfortunately, thermal storage systems don’t have a one-size-fits-all approach. One thermal battery chemistry that stores heat at 100° C, for instance, won’t be able to serve the market for heat at ~1000° C. And while you can go the other way around (i.e., from 1000° C → 100° C), you suffer from efficiency loss, which impacts applicability.
We’re seeing market tailwinds to decarbonize the heat industry. As environmentally-conscious companies reduce their Scope 1, 2, and 3 emissions, they’re applying pressure on their supply chains to decarbonize. For example, Apple is committing to 100% carbon neutrality for its supply chain. This means that Apple’s suppliers for glass, steel, plastics, and other materials must find sources of zero-carbon heat.
The recently-passed Inflation Reduction Act (IRA) provides further push to decarbonize industrial heat. For example, the IRA includes a ~$6 billion pool in Department of Energy (DOE) competitive grants for heavy industries to invest in technologies that accelerate greenhouse gas emissions reductions (aka the Advanced Industrial Facilities Deployment Program). The IRA also provides $10 billion in tax credits for investing in the buildout of qualifying energy technologies, which include storage modalities like thermal storage and other technologies to provide zero-carbon heat.
As we mentioned, the market for thermal storage goes beyond just heat. Thermal batteries can also supply electricity (since heat can be converted to electricity) and take a share of the long-duration energy storage market (LDES).
It’s easiest to think about the importance of LDES in the context of renewable solar energy. Because solar energy is intermittent (since the sun doesn’t shine at night), there’s a supply/demand imbalance for renewable solar. At peak hours like noon, the grid is flooded with an abundant supply of cheap solar energy, but at night, there’s no renewable solar energy available. Enter LDES, which can store the excess solar energy during the day, and rapidly deploy it during the night to “smooth” solar output.
The difficult aspect with evaluating LDES, though, is the number of different technologies available. Today, lithium ion batteries like those powered by our portfolio company Sila Nanotechnologies are king in terms of technological readiness and deployability. However, lithium-ion batteries still suffer from long-term degradation, short storage durations, and supply chain shortages. So, there is a gap in the storage market for LDES to fill. The question for thermal batteries is whether they can economically compete with other forms of storage for 12+ hours. These other competitors include technologies like pumped hydro storage (i.e., using energy to pump water up a hill and releasing the water down the hill to generate electricity when needed) or compressed air storage (i.e., using energy to compress air in an underground cavern and releasing the air to generate electricity when needed).
Thermal storage has a few advantages:
- Components: Thermal batteries rely on conventional components, like heat exchangers and compressors, that are already widely used in the power and processing industries, meaning the facilities are easier and quicker to build than many alternatives.
- Location: Thermal storage plants can be deployed in more locations and can be scaled up to meet the grid’s storage requirements. Other LDES technologies are limited to specific geographies: pumped hydro requires mountains and valleys able to hold vast reservoirs, and compressed air energy storage is dependent on large subterranean caverns.
- More input sources: Thermal batteries can be charged not only with excess electricity from the grid, but also from heat sources like waste heat from industrial processes. While other LDES technologies can theoretically also store heat in the form of usable energy, they need to convert such heat to other forms of energy, which creates an efficiency loss.
How does thermal energy storage work?
If you squint, you can think of the ocean as one giant thermal battery. Due to water’s chemical properties, it requires a large amount of heat to raise its temperature (i.e., water has a high “specific heat”). So, during the day, the ocean will absorb a significant amount of heat from the sun without much warming. At night, the ocean releases the heat absorbed during the day, and sea breeze carries the warm air inland to the coast. This is why coastal cities tend to have more moderate climates.
Thermal batteries work similarly. In broad strokes, a thermal storage system must have three things. First, like the ocean, a thermal storage system must have some material or fluid to absorb and retain heat. This storage can come in one of three forms: sensible, latent, and thermochemical.
- Sensible heat storage is the most straightforward: A material or fluid stores thermal energy and increases in temperature. While sensible heat storage can deliver temperatures north of 1000 C, heat discharge rate can be limited and inefficient.
- Latent heat storage is when a material or fluid stores thermal energy but does not increase in temperature because the material is going through a phase change (e.g., solid to liquid or liquid to gas). Latent heat storage materials can be more efficient in storing and discharging heat than sensible storage materials but only serve a narrow range of temperatures and cannot reach > 1000 C.
- With thermochemical storage, a compound (say, AB) absorbs energy and is converted chemically into two compounds (say, A and B). In the reverse reaction, A and B are combined back to form AB, releasing heat. While thermochemical storage enjoys high efficiency, this is an area that’s still being explored.
Second, the system must have an energy source to “charge” the material. This can come from concentrated solar power, nuclear heat, electricity converted to heat, heat offtake from industrial processes, etc. Companies building thermal batteries face the challenge of finding a cheap, abundant source of heat to charge their batteries.
Third, the system must have a way to discharge the heat, typically through convection (passing a heat exchange medium like air through the thermal battery to carry the heat). Another way to discharge heat is through radiation, as hot objects will naturally emit energy in the form of photons (e.g., this is how the Sun heats the Earth). Transporting heat, however, is dangerous, so thermal batteries often need to be co-located with the end user of the heat or converted electricity.
Thermal batteries can similarly be used for cooling. The main difference is that the heat coming in must first be converted to electrical energy that is used to cool the storage medium. This conversion from heat to electricity results in efficiency loss. The rest of the process is roughly the same. When a building needs to be cooled, a heat exchange medium passes through the thermal storage system and carries cold air to the end destination.
Three generations of thermal storage technologies
We’ve been through three generations of thermal storage technologies. The first generation featured oils and water as the heat storage fluid. High specific heat, chemical stability, and low cost made these working fluids a good storage media. Furthermore, these fluids are widely available and well-understood, making them a clear choice for the first generation of TES. However, because these liquids have low boiling points (~100-250 C), they are typically more suitable for low-temperature applications like residential heating and cooling.
In the 1980s and 1990s, second generation thermal storage featured molten salts. Thanks to concurrent experiments with molten salts in nuclear reactors, researchers and developers also began to play around with molten salts for TES. Molten salt thermal batteries can deliver heat at higher temperatures than can first-gen TES, as molten salts melt around 280° C and can stay molten up to ~600° C before decomposing. For example, one of the leaders in molten salt thermal storage is Malta, which can store heat at temperatures of ~565° C. Today, much of the research into molten salts is developing new molten salts that can go to higher temperatures (e.g., magnesium fluoride can be taken up to 800° C). The problem with molten salts, however, is that they are corrosive to the storage medium, which means higher labor and materials costs to maintain the system.
We’re now entering the third generation of thermal storage systems, which has largely been driven not by new research breakthroughs or innovation, but by increasing environmental, social, and governance (ESG) and market tailwinds to decarbonize industrial heat. The dominant technology for this third-generation of TES has been particle-based thermal storage, which stores heat with a big tank of hot sand or engineered bricks. These materials are relatively cheap, and the operating temperature range can go to > 1000° C, making them better candidates to decarbonize industrial heat than first-or second-gen TES. However, the problem with such high temperatures is that you’ll need to house the storage materials in containers that have enough thermal stability. You often need expensive superalloys, which can be > 100x more expensive than stainless steel, and you sometimes need to house these materials in inert gasses that won’t react with the material at such high temperatures. Many of the new startups in the TES space are building particle-based storage like Antora, Electrified Thermal, Rondo, KraftBlock, MGA Thermal, and EnergyNest.
Evaluating thermal storage
The best thermal batteries can store a significant amount of heat with minimal loss as heat is transferred and stored. So, when evaluating any thermal storage system, it’s important to examine the following factors:
1. Target market
What’s the use case? Is the target market industrial heat or LDES? It’s also important to remember that supplying both industrial heat and LDES is typically part of a brownfield, not greenfield, value chain. Thermal batteries often need to be co-located near industrial sites/utilities, integrate within existing facilities, or take over retired facilities. Therefore, it’s also important to ask whether a target customer geography or facility can support the deployment of a TES system.
2. Materials
What is the material used to store the heat, what are its specific thermodynamic properties, and what is the supply chain for that material? These factors depend on the chemical properties of the material used to store the heat, the heat exchange carrier, and how the materials are insulated. For reference, the more rudimentary TES systems using water as a heat storage medium have an energy density of ~10 kWh/m3 and a power of 1 KW. More advanced TES systems today have a capacity and density of ~100 kWh/m3 and power of > 100 KW.
3. Storage period
How long will the stored heat last (i.e., hours, days, weeks, or months)? This is especially important for the LDES market, where solar and wind energy is intermittent (as discussed). For example, we’ll need longer-duration LDES during the winter months, when we get less sunlight.
4. Cost
What are the unit economics? One metric, the levelized cost of storage/heat (LCOS/LCOH), is the total lifetime cost of energy storage technology divided by its cumulative delivered electricity/heat. These metrics tell you what the discharged electricity/heat would need to be sold for in order to break even on the overall storage lifecycle costs (capex and opex) across its life. While you need to be careful about using LCOS/LCOH as comparison metrics, they can provide a rough apples-to-apples comparison with other forms of electricity/heat delivery if you isolate a specific use case and duration. For reference, lithium-ion batteries currently have a LCOS of ~$200/MWh for 2- to 4-hour charge and discharge times.Another cost metric to keep tabs on is installed cost, which is total capital costs per unit of energy to develop and integrate a storage system into a system. We expect thermal storage LCOS/LCOH and installed cost to come down rapidly thanks to cheaper materials, more developed supply chains, and economies of scale with larger facilities. The installed cost of various energy storage systems is shown below. Lithium-ion batteries will continually outperform other technologies for short-duration, low-power use cases, but we expect thermal batteries to go down the cost curve and become cost-competitive with other LDES methods.
One other metric is round-trip efficiency (RTE). Energy storage typically consumes energy, saves it in some manner, then hands it back to the grid. The ratio of energy-in to energy-out is the RTE. All else equal, lower RTE results in poorer unit economics. According to thermodynamics, any conversion of energy from one form to another creates waste. Thermal storage systems today have a relatively modest 50–70% conversion efficiency to electricity. Compare this efficiency with 80–90% for lithium-ion batteries or 70–85% for pumped hydro power. However, thermal batteries can achieve higher RTE with higher discharge temperatures and have a 95%+ RTE for heat (i.e., no conversion to electricity).
The thermal storage market is hot
Thermal storage holds promise as a way to decarbonize industrial heat and store energy from the grid, both of which are enormous markets. The urgent need for TES mainly stems from market tailwinds, and indeed, we’re excited for the technology to take off. The physics and chemistry behind thermal storage are well-understood, the systems needed for TES are widely available, and although TES companies are engineering new compounds to store heat, the supply of materials for these compounds is relatively unconstrained. The remaining challenges for TES are engineering and sales, not fundamental science or dwindling supply.
However, thermal storage is only one technology among an entire ecosystem of heating, cooling, and storage solutions, which include solutions like nuclear, heat pumps, geothermal, and forms of mechanical and chemical storage. As thermal storage companies continue to build, we’re excited for thermal batteries to increase in adoption and go down the cost curve.
If you’re building a thermal storage company, we’d love to chat! Email our team at climate@bvp.com.